Hinode, IRIS, and ATERUI Cooperate on 70 year old Solar Mystery -Magnetically driven resonance helps heat the Sun's atmosphere!-
Naional Astronomical Observatory of Japan (NAOJ)
Institute of Space and Astronautical Science / Japan Aerospace Exploration Agency (ISAS/JAXA)
Nagoya University
National Aeronautics and Space Administration (NASA)
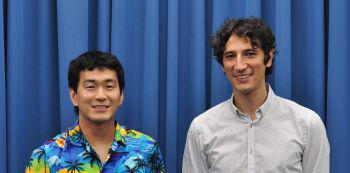
Solar physicists have captured the first direct observational signatures of resonant absorption, thought to play an important role in solving the "coronal heating problem" which has defied explanation for over 70 years.
An international research team from Japan, the U.S.A., and Europe led by Drs. Joten Okamoto and Patrick Antolin combined high resolution observations from JAXA's Hinode mission and NASA's IRIS (Interface Region Imaging Spectrograph) mission, together with state-of-the-art numerical simulations and modeling from NAOJ's ATERUI supercomputer. In the combined data, they were able to detect and identify the observational signatures of resonant absorption.
Resonant absorption is a process where two different types of magnetically driven waves resonate, strengthening one of them. In particular this research looked at a type of magnetic waves known as Alfvénic waves which can propagate through a prominence (a filamentary structure of cool, dense gas floating in the corona). Here, for the first time, researchers were able to directly observe resonant absorption between transverse waves and torsional waves, leading to a turbulent flow which heats the prominence. Hinode observed the transverse motion and IRIS observed the torsional motion; these results would not have been possible without both satellites.
This new information can help explain how the solar corona reaches temperatures of 1,000,000 degrees Celsius; the so called "coronal heating problem."
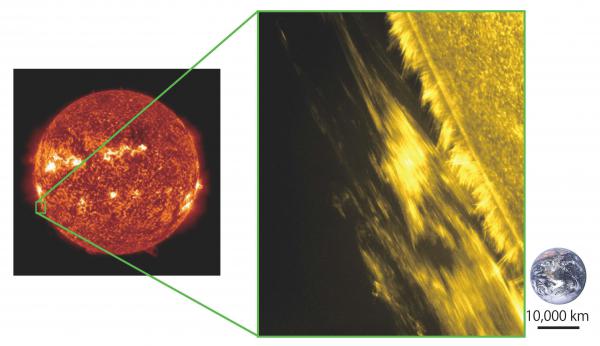
The solar corona, the outer layer of the Sun's atmosphere, is composed of extreme high temperature gas, known as plasma, with temperatures reaching millions of degrees Celsius. As the outer layer of the Sun, the part farthest from the core where the nuclear reactions powering the Sun occur, it would logically be expected to be the coolest part of the Sun. But in fact, it is 200 times hotter than the photosphere, the layer beneath it. This contradiction, dubbed "the coronal heating problem" has puzzled astrophysicists ever since the temperature of the corona was first measured over 70 years ago.
Space-borne missions to observe the Sun and other technological advances have revealed that the magnetic field of the Sun plays an essential role in this riddle. But the key to solving the "coronal heating problem" is understanding how magnetic energy can be converted efficiently into heat in the corona. There have been two competing theories.
The first theory involves solar flares. Although each flare converts large amounts of magnetic energy into thermal energy, the overall frequency of solar flares is not high enough to account for all of the energy needed to heat and maintain the solar corona. To solve this discrepancy, the idea of "nanoflares" was introduced. It is thought that nanoflares, miniature solar flares, occur continuously throughout the corona and that the sum of their actions convert enough magnetic energy into heat to make up the difference. Unfortunately, such nanoflares have yet to be detected.
The second hypothesis is based on magnetically driven waves. Thanks to space missions such as the Japanese "Hinode" mission (launched in 2006), we now know that the solar atmosphere is permeated with "Alfvénic" waves. These magnetically driven waves can carry significant amounts of energy along the magnetic field lines, enough energy in fact to heat and maintain the corona. But for this theory to work, there needs to be a mechanism through which this energy can be converted into heat.
To look for this conversion mechanism, the research team combined data from two state-of-the-art missions: Hinode and the IRIS imaging and spectroscopic satellite (the newest NASA solar mission, launched in 2013).
Both instruments targeted the same solar prominence (see Figure 1). A prominence is a filamentary bundle of cool, dense gas floating in the corona. Here, 'cool' is a relative term; a prominence is typically about 10,000 degrees. Although denser than the rest of the corona, a prominence doesn't sink because magnetic field lines act like a net to hold it aloft. The individual filaments composing the prominence, called threads, follow the magnetic field lines.
Hinode's very high spatial and temporal resolution allowed researchers to detect small motions in the 2-dimensional plane of the image (up/down and left/right). To understand the complete 3-dimensional phenomenon, researchers used IRIS to measure the Doppler velocity (i.e. velocity along the line of sight, in-to/out-of the picture). The IRIS spectral data also provided vital information about the temperature of the prominence.
These different instruments allow the satellites to detect different varieties of Alfvénic waves: Hinode can detect transverse waves while IRIS can detect torsional waves. Comparing the two data sets shows that these two types of waves are indeed synchronized, and that at the same time there is a temperature increase in the prominence from 10,000 degrees to more than 100,000 degrees. This is the first time that such a close relationship has been established between Alfvénic waves and prominence heating.
But the waves are not synchronized in the way scientists expected. Think of moving a spoon back-and-forth in a cup of coffee: the half-circular torsional flows around the edges of the spoon appear instantly as the spoon moves. But in the case of the prominence threads, the torsional motion is half-a-beat out of sync with the transverse motion driving it: there is a delay between the maximum speed of the transverse motions and the maximum speed of the torsional motion (see Figure 2), like the delay between the motion of the hips of a dancer in a long skirt and the motions of the skirt hem.
To understand this unexpected pattern the team used NAOJ's ATERUI supercomputer to conduct 3D numerical simulations of an oscillating prominence thread. Of the theoretical models they tested, one involving resonant absorption provides the best match to the observed data. In this model, transverse waves resonate with torsional waves, strengthening the torsional waves; similar to how a child on a swing can add energy to the swing, causing it to swing higher and faster, by moving his body in time with the motion. The simulations show that this resonance occurs within a specific layer of the prominence thread close to its surface (see Figure 3). When this happens, a half-circular torsional flow around the boundary is generated and amplified. This is known as the resonant flow. Because of its location close to the boundary, the maximum speed of this flow is delayed by half-a-beat from the maximum speed of the transverse motion, just like the pattern actually observed (see Figure 2).
The simulations further reveal that this resonant flow along the surface of a thread can become turbulent. The appearance of turbulence is of great importance since it is effective at converting wave energy into heat energy. Another important effect of this turbulence is to enlarge the resonant flow predicted in the models to the size actually observed.
This model can explain the main features of the observations as the results of a two-step process. First resonant absorption transfers energy to the torsional motions, producing a resonant flow along the surface of the prominence thread. Then turbulence in this strengthened resonant flow converts the energy into heat (see Figure 2).
This work shows how the power of multiple satellites, such as Hinode and IRIS, can be combined to investigate long-standing astrophysical problems and will serve as an example for other research looking for similar heating in other solar observations.
These results were published in The Astrophysical Journal, Vol 809 in August, 2015.
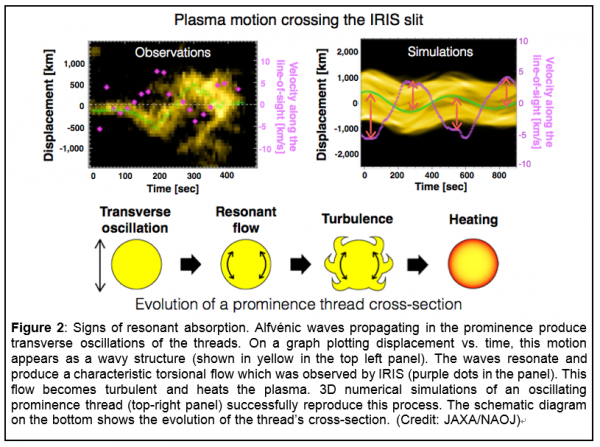
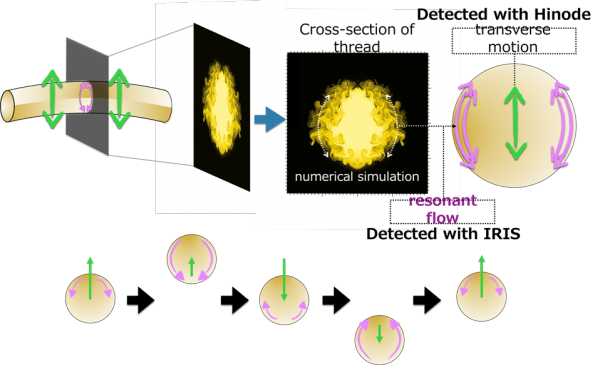
More details are shown below.
Hinode, IRIS, and ATERUI Cooperate on 70 year old Solar Mystery
-Magnetically driven resonance helps heat the Sun's atmosphere!-
A group of solar physicists from Japan, USA and Europe have joined efforts to directly detect for the first time a long hypothesized heating mechanism in action in the solar corona, the outer layer of the solar atmosphere.
By combining high resolution observations from JAXA's Hinode mission and NASA's IRIS mission, together with state-of-the-art numerical simulations with NAOJ's ATERUI supercomputer, the group, lead by Drs. Joten Okamoto and Patrick Antolin, has managed to detect and identify the observational signatures of resonant absorption. This wave-related mechanism of energy conversion is thought to play an important role in the so-called "coronal heating problem", one of the most important unsolved astrophysical problems for mankind.
The group explains in a pair of papers in the The Astrophysical Journal, published on August 11th, how the mechanism of resonant absorption can lead to significant heating in the solar corona. Magnetic (Alfvénic) waves propagating in structures in the solar atmosphere (a prominence in this case) produce up/down transverse oscillations of the threads. The waves resonate and produce a characteristic flow which becomes turbulent and heats the plasma. The imprint of this flow is detected thanks to the combination of Hinode and IRIS.
Credit: NASA/JAXA/NAOJ
The problem
Many unsolved astrophysical mysteries still surround the Sun, our closest star, and extrapolate to most of the stars in the Universe. Among all these mysteries there is one which has puzzled astrophysicists for over 70 years, extremely important to mankind since it characterises the space environment surrounding the Earth. The solar corona, which can be observed with our own eyes during solar eclipses, is the outer layer of the solar atmosphere and is characterized by extremely high temperatures of several million degrees. The surprisingly high temperatures of the coronal material (known as plasma) contrast even further with the temperatures of the photosphere, the surface of the Sun: despite being closer to the source of energy the photosphere is 200 times cooler than the corona. The advent of space missions and technological advance have shown that the magnetic field of the Sun plays an essential role in this riddle, and that the solution resides in understanding how to efficiently convert magnetic energy into thermal energy in stellar atmospheric plasmas. The unknown mechanisms are especially complicated since stellar plasmas are extremely tenuous and dissipation processes leading to heat are difficult to achieve.
The usual suspects
There are two main candidates for heating the solar corona. The first one is related to flares, those spectacular energy releases in the solar atmosphere that can now easily be seen in X-ray observations of the Sun. Such phenomena involve large scale magnetic to thermal energy conversion in a process known as magnetic reconnection (magnetic field lines undergo a rapid restructuring process leading to different connectivity and significant energy release), but are however not frequent enough to heat the entire solar corona. A leading theory for explaining the solar corona is based on this mechanism, but at much smaller energetic scales. Known as the "nanoflare" (-reconnection) theory, it stipulates that reconnection may be occurring everywhere in the corona, leading to energy release in spatial locations too small to be detected observationally but frequent enough to be effective.
The other main competing theory is based on magnetic waves (known as MHD - magnetohydrodynamic - waves). Thanks to space missions such as the Japanese "Hinode" mission (launched in 2006), now we know that the solar atmosphere is permeated with such waves. Of particular importance among magnetic waves are "Alfvénic" waves, which can carry significant amounts of energy along the magnetic field lines, enough to heat and maintain a corona. For over 30 years scientists have hypothesized on a mechanism of dissipation of these waves for heating the plasma. It is not enough to be able to carry this energy. For this theory to be successful it needs a mechanism that is able to dissipate the wave energy and heat the plasma. An essential part of this process is "resonant absorption".
Resonant absorption has the ability to concentrate large quantities of wave energy into small spatial locations (see Appendix for details). In simple words, this mechanism involves resonance between 2 different kinds of Alfvénic waves(*1). However, the concentrated energy from resonant absorption needs to find a second mechanism that efficiently dissipates the energy away and heat the plasma. Furthermore, this mechanism involves a complicated 3D motion of the plasma, something that has never been detected, until now.
(*1)The traditional "Alfvén" wave and the transverse MHD wave (also known as kink wave)
Credit: JAXA/NAOJ
A powerful duo: Hinode and IRIS
The group solved this problem by combining two powerful instruments: The SOT (Solar Optical Telescope) imaging telescope on board of the Hinode satellite, and the IRIS imaging and spectroscopic satellite (the newest NASA solar mission, launched in 2013). A particularity of Hinode is its very high spatial and temporal resolution, allowing to detect small plasma motions in the plane-of-the-sky (up/down and left/right motions from our view-point). A particularity of IRIS is to be able to measure at high resolution the motion along the line-of-sight (known as Doppler velocity, i.e. motion that comes towards or goes away from us). Furthermore, these two instruments can detect light produced under different temperature conditions. The combination of both instruments therefore allowed a tomographic scan of a specific region of the Sun, providing not only the 3D motion but also the temperature evolution of the plasma.
The target for both instruments is a solar prominence (see Figures 1 and 2). Prominences are puzzling structures in the solar corona, composed of cool material around 10,000 degrees, 100 times cooler than the surrounding plasma of the corona. Prominences can be very large, spanning a few hundred thousand kilometres (more than 10 times the Earth diameter), but are composed of small thread-like substructure that are called prominence threads (of only a few hundred kilometres in width).
The imprint of resonant absorption
In 2006 Hinode discovered that prominence threads are constantly oscillating transversely, that is, sideways. The observations with IRIS revealed that the threads also oscillate along the line-of-sight and are synchronized with their sideways displacements in a very special way. When the plasma attains a maximum sideways displacement (for instance, maximum up or down position), it comes towards us or goes away from us at the fastest speed (see Figure 3). On Earth this is not expected. For example, if you move your coffee spoon sideways, the coffee will instantaneously flow around the spoon (in a half-circular motion). The maximum velocity of the coffee then occurs when the sideways displacement of the spoon is halfway through the movement.
A scenario in which the peculiar prominence motion can be imagined is that of a dancer with a long skirt (see Figure 4). The left - right hip motions of the dancer are analogous to the up and down motions of the prominence plasma detected by Hinode. The circular motions of the skirt are analogous to the motions along the line-of-sight detected by IRIS. As in the observations, the skirt can move past the dancer at maximum speed when her hips have stopped moving.
Hinode and IRIS also detected several signatures of heating within the prominence, with temperatures increasing from 10,000 degrees to at least 100,000 degrees, suggesting that the waves had an important role in the heating of the plasma, and thus also in coronal heating (see Figure 6).
Credit: JAXA/NAOJ
Credit: NAOJ
Credit except "The great wave off Kanagawa": NAOJ
Credit: JAXA/NAOJ
Observations explained: coupling between two mechanisms
In order to understand the peculiar motion and heating of the prominence threads the group carried out advanced numerical simulations in 3D with NAOJ's ATERUI supercomputer of an oscillating prominence thread.
As the thread oscillates up and down resonant absorption sets-in. As explained in the Appendix, this mechanism involves a phenomenon of resonance between Alfvénic waves. Similar to the coordinated motion of a person on a swing, in order to increase the amplitude of the swing, Alfvénic waves can have coordinated motions (and thus resonate) leading to an increase of amplitude. This occurs in a specific layer within the prominence thread, close to its boundary (see Figure 4). When this happens an amplified half-circular flow is generated (the resonant flow) around the boundary, matching very well the observed coordinated motion (see Figure 3).
The simulations further reveal that the special resonant flow generated by the waves becomes turbulent, producing a myriad of vortices, similarly as strong winds can generate sea waves (the vortices are produced by a dynamic instability known as the Kelvin-Helmholtz instability, see Figure 5). The generation of turbulence (vortices) is of great importance since it leads to significant dissipation of the wave energy through currents and friction.
Another important effect of this turbulence is to enlarge the resonant flow, which gets heated. The flow carries the momentum and the energy from the small locations of the resonance over larger spatial scales, thereby ensuring significant heating and detectability with the current instrumentation (Hinode and IRIS). The proposed model, which shows how resonant absorption and turbulence can couple to efficiently heat the plasma, can therefore explain the main observed features.
Paving the way
This work provides for the first time strong evidence of a potential coronal heating mechanism and takes us one step closer towards solving the coronal heating problem. This work further serves as a pathfinder to search for similar heating in other solar observations and shows how the power of multiple satellites such as Hinode and IRIS can be combined to provide solutions to long standing astrophysical problems.
APPENDIX
Resonant absorption: a long hypothesized mechanism for coronal heating
Resonant absorption is an efficient mechanism for energy conversion of waves. As the name dictates, the mechanism is based on the phenomenon of resonance. Like with a person in a swing, the coordinated motion between Alfvénic waves can lead to an increase of amplitude of the waves. In the case studied here it is 2 kinds of waves that resonate with each other. Both belong to the family of Alfvénic waves but they differ because one is a global wave, funnelled along the prominence thread (named transverse MHD wave or kink wave since it is able to swing the thread transversely) while the other is a local wave and varies from place to place (and is just called Alfvén wave or torsional Alfvén wave when it is inside the thread since they produce torsional motions; they are not able to move the entire thread structure).
A necessary condition for the resonance between these two waves to take place is that they must travel at the same speed. While the speed of transverse MHD waves is fixed by the global details of the thread and its environment, the speed of the (torsional) Alfvén wave can change from location to location inside the thread, depending on the local density. The smaller the local density the larger the speed of these waves. As Figure 7 shows the speed can change drastically from the center of the thread to the exterior. This change implies that there is a layer close to the boundary of the thread where the speeds of both waves will be the same. This is where resonance occurs. It is expected that this resonance layer is very small and cannot be directly detected by current instruments. Due to the cylindrical shape of threads the resonant flow produced by the waves will show a half-circular (torsional) motion. As in the case of the swing, where kinetic energy from the person is transferred to half-circular motions of the swing, which become amplified, in the thread the transverse MHD wave passes its energy to the torsional wave in the resonance layer (which produces the resonant flow that is detected in this work). The resonance therefore converts transverse motions of the entire thread (the up and down motions from the transverse MHD waves, analogous to the dancer's hip motions) into local half-circular (torsional) motions at the boundary of the threads (which we compare to the half-circular motions the skirt of the dancer makes).
Resonance actually occurs over a small spatial width around the point of same wave speeds. Since the Alfvén speed increases the farther we are from the prominence center, the resonant (half-circular) flows within this resonant layer around the boundary will occur at slightly different times depending on the distance from the center. The further we are from the center, the faster the resonant flow will occur (a process known as "phase mixing"). The eventual consequence of this is to produce the observed peculiar motion between the up and down transverse motion (detected with Hinode) and the half-circular motion (detected with IRIS): the maximum speeds along the line-of-sight occur roughly at times of maximum up and down transverse displacement.
Credit: NAOJ
Papers
"Resonant Absorption of Transverse Oscillations and Associated Heating in a Solar Prominence. I. Observational Aspects"
T. J. Okamoto, P. Antolin, B. De Pontieu, H. Uitenbroek, T. Van Doorsselaere, T. Yokoyama 2015, The Astrophysical Journal, 809, 71
"Resonant Absorption of Transverse Oscillations and Associated Heating in a Solar Prominence. II. Numerical Aspects"
P. Antolin, T. J. Okamoto, B. De Pontieu, H. Uitenbroek, T. Van Doorsselaere, T. Yokoyama 2015, The Astrophysical Journal, 809, 72
Acknowledgements
This work was supported by JSPS KAKENHI grant No. 25220703 (PI: S. Tsuneta) and JSPS KAKENHI Grant No. 25800120 (PI: T. J. Okamoto). Numerical computations were carried out on "ATERUI" (Cray XC30) at the Center for Computational Astrophysics, NAOJ. This work was also (partly) carried out on the Solar Data Analysis System, operated by the Astronomy Data Center in cooperation with the Hinode Science Center of the National Astronomical Observatory of Japan.
Regarding the use of images and movies on the page concerned, please visit the page here. The credits of images and movies on this page are “NAOJ/JAXA” unless explicitly stated to the contrary. Regarding images and movies on this page the credits of which are “NAOJ/JAXA”, "NAOJ/JAXA/MSU", or “NAOJ, JAXA, NASA/MSFC”, terms of use for Copyrighted Works owned by NAOJ can be applied. In using the images and movies, the credits should be given.